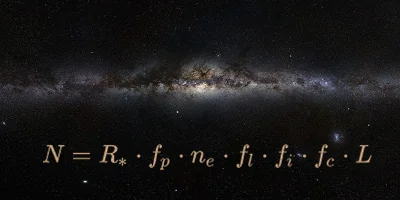
How many technological civilizations exist in the Milky Way? What the origin of life on Earth tell us about the parameters of the Drake equation.
Under good conditions, you can see 6000 stars in the night sky with the naked eye. This is our immediate cosmic neighborhood and only a vanishingly small part of the Milky Way. The much larger part can be seen from Earth only as a bright band in the night sky. The individual stars are too far away to be perceived individually. But even this is only a small part of a galaxy with so many stars that we can't even tell to within 150 billion how many there are. Estimates range from 100 to 400 billion. Most of them will remain hidden from us forever behind dust clouds and countless other stars.
The diameter of the Milky Way is 185000 light years. If it would be possible to observe the earth at this time from a planet on the other side of the galaxy, then one would see a planet with mighty polar caps. The ice far advanced into middle latitudes. A planet inhabited, among others, by two humanoid species. The Homo Sapien and the Neanderthal man. It would be the light of a time long past.
If there are so many stars and life could arise on Earth, why not elsewhere in the Milky Way? How many other civilizations could exist? It is a question that also interested the American astrophysicist Frank Drake. In 1961 he presented an equation with which the number of technical, intelligent civilizations in the Milky Way can be estimated.
This equation, named after him, reads:
\begin{equation} \Large \nonumber N = R_* \cdot f_p \cdot n_e \cdot f_l \cdot f_i \cdot f_c \cdot L \end{equation}Where:
Currently, we only have a relatively good idea of the magnitude of the first two parameters of the equation (\(R_*\) and \(f_p\)). All other values are not even rudimentarily known. As a result of this uncertainty, one should not really ask for the result of the Drake equation at all. The result is whatever you want it to be. You want a galaxy with many civilizations (N>100000) like in Gene Roddenberry's Star Trek? No problem, there are suitable parameters for it. You want to be alone in the Milky Way, maybe even in the universe (N<<1)? No problem, there is also a parameter set for it. According to our present knowledge, no parameter set is better or worse than the other. However, the desire for numbers is understandable and no one wants to read an article about the Drake equation that does not present a result, and so I too will present two estimates for the result of the equation at the end of this article.
The real utility of the equation is not in calculating a numerical value. In the 60+ years since its formulation, countless research papers have been published dealing with the equation and estimates for its parameters. It was certainly on the minds of the astronomers who discovered the first exoplanets 30 years ago, and perhaps even a driving factor for some of them. No equation has stirred the imagination and discussion on the subject of the search for extraterrestrial life more than the Drake equation. So when NASA is searching for microfossils on the red planet with the Mars rover Perseverance, the Drake equation is always present as any such research will help to determine the parameters of the Drake equation more precisely.
At what rate do stars form in whose planetary systems life could evolve? To clarify this, we should find out what the requirements are for a star to make life possible. The problem with this is that Earth is the only example we know of a planet where intelligent life has arisen. So let's begin with an excursion into Earth's history.
An important factor in assessing the suitability of a star for the emergence of intelligent life is its lifetime. The star must be able to maintain a stable habitable zone for long enough to allow the initial development of life and its evolution into intelligent life.
Earth is 4.54 billion years old. Different studies date the beginning of the life on the basis of microfossils respectively their leftovers to the time before 3.5 - 4.1 billion years [2]. On a geological time scale, life on Earth originated early. Just 300 million years after the formation of a first solid earth crust. At least on Earth, it appears that simple life arose as soon as the right environmental conditions were present. These include a solid earth crust, and the presence of liquid water.
The first life forms were simple single-celled organisms without a nucleus, called prokaryotes. Bacteria and archaea belong to this group. The latter, formerly also called primordial bacteria, are specialized in habitats where extreme conditions prevail. They are still found today in geysers and in the vents of the so-called "black smokers" in the deep sea. Some species can survive without oxygen and use molecular hydrogen for their metabolism instead.
Life on Earth may have arisen quickly, but it remained single-celled for more than 2 billion years. That is an amazingly long period of time. Then, about 1.6 to 2.1 billion years ago, eukaryotes emerged. Initially simple single-celled organisms with more complex cell structures. With a diameter of 10 to 30 micrometers, the cells are much larger than those of the prokaryotes. They subdivide into cell organelles, which perform various functions similar to the organs of the human body. A nucleus contains the main part of the genetic material, which comes in the form of DNA.
The evolution from unicellular to multicellular organisms took place independently at least 25 times in the group of eukaryotes. All known plants, animals and fungi on Earth belong to the group of eukaryotes. The time span from their emergence to the emergence of intelligent life was another 2 billion years. Altogether the evolution needed more than 4 billion years until highly developed life forms could complete the last step towards universal intelligence.
The evolution to intelligent life is a multistage process, which probably needs several billion years, should it take place at all. On Earth, the evolution from nucleated prokaryotes to more complex eukaryotes took about as long as the evolution from unicellular eukaryotes to humans. All land life forms known today evolved only in the last 500 million years. Stars that allow the evolution of intelligent life should therefore have a lifetime of at least several billion years. At present it is assumed that in the Milky Way per year 1.5 - 3 such stars are newly formed. Their mass is on average half the mass of the sun.
$$\Large 1.5 < R_* < 3.0$$
The history of exoplanet discoveries is still young. The earliest discoveries were made in the late 1980s. Today, the list of confirmed exoplanets contains over 4000 entries and new ones are added every year.
The methods of exoplanet discovery are diverse, but they come up against physical limits. At present we can often detect exoplanets only when we look at the edge of their planetary system. Moreover, the two most successful detection methods, the transit method and the radial velocity method, favor large planets in close proximity to their central star.
Thus, many planetary systems remain hidden from us, but where physical observability leaves gaps, statistics help us fill them. For each discovered planetary system we can assume that a certain number of planetary systems is not observable with our present technology. Thus, despite incomplete data, we can estimate the total number of planetary systems. Recent research [4] based on microlensing events suggests that planetary systems are the rule and the value of \(f_p\) could be close to one.
$$\Large f_p \approx 1$$
In order to estimate the average number of life-friendly planets per solar system, one should first examine the requirements for such a planet. Presently we can only make assumptions which seem plausible based on the composition of the solar system and the history of the earth.
A first requirement is that it is a rocky planet. Gas giants have no solid surface and a dense atmosphere consisting essentially of hydrogen and helium. In addition, high wind speeds and a strong radiation belt make microbial life highly unlikely. In short, without a solid planetary surface, it becomes very difficult for higher life to emerge.
The next condition for the emergence of life is the existence of liquid water. It serves as a solvent and transport medium and facilitates the chemical reactions necessary for the formation of the building blocks of life. Without water, even the emergence of the simplest microbial life is difficult to imagine. The area in a planetary system where liquid water can exist is called the "habitable zone."
Evolution is a process by which life can adapt to changing environmental conditions. The slower the change, the more successful it is. For this reason, the planet's orbit should be stable. It should remain in the habitable zone for a very long time. For the Earth, this has been the case for over 4 billion years. Computer simulations show that this orbital stability cannot be taken for granted.
The rotation axis should also be stable, because fluctuations in the rotation plane would quickly lead to extreme climate changes on the planet. These could also make the planet uninhabitable, even though it is in the habitable zone. An example for this would be, a shift of the rotation plane in a way that a part of the planet would lie in permanent darkness.
Other factors can only be conjectured. Is a geologically active planet necessary, since only such planets can maintain a strong magnetic field? Should the planet have a moon that would stabilize the axis of rotation and create tides that would help life colonize the land? Is a large gas planet in the planetary system necessary to protect life on the inner planets from extinction by too many asteroid impacts? All these circumstances make it very difficult to estimate \(n_e\) directly.
After observations of exoplanets with the Kepler Space Telescope, researchers estimate that there may be 40 billion Earth-like planets in the Milky Way in the habitable zone of Sun-like stars or red dwarfs. This suggests that the product of \(f_p\) and \(n_e\) is about 0.4:
$$\Large f_p * n_e \approx 0.4$$
This parameter deals with the question of how likely it is that life will actually develop if the planet makes it possible in principle. Here we are talking about microbial life, so-called prokaryotes, the simplest single-celled organisms. A form of life that still exists on Earth today in the form of bacteria and archaea. The only certainty is that the parameter must lie between 0 and one.
$$\Large 0 < f_l < 1$$We do not have enough information to determine this parameter but a look at the history of Earth shows that life developed rather quickly. The earth cooled down, the cosmic bombardment with asteroids stopped and after, in geological time spans short, 300 million years the first primitive unicellular organisms emerged. This could have been coincidence, however, it could also indicate that \(f_l\) is close to one and there is a good chance to confirm this in the future.
If unicellular life arises comparatively fast, then this should have happened several times in parallel on the primordial Earth. This could be proven by finding bacteria that are not genetically related to any other life form on Earth. So far, this has not been successful, but it could also be due to the fact that in the end one type of microbial life prevailed and displaced all others.
But Earth is not the only place in the solar system where life is conceivable. Mars and Earth were more similar in their early days than they are today. It is assumed that Mars also had oceans, a denser atmosphere, active volcanism and a magnetic field 4 billion years ago. Except for size, the two planets were probably very similar during the first 500 million years. If microbial life could form on Earth, why not on Mars?
Later, Mars cooled, lost its magnetic field, large parts of its atmosphere, the surface water froze, and higher life probably never could develop. But if the formation of unicellular life is indeed rapid, perhaps fossil traces of it could be found on Mars as well.
The same applies also to the Jupiter moon Europa, under whose mighty ice layers life could have formed in an underlying ocean. The energy source there would not be the sun, but the volcanism of the moon caused by tidal friction.
The emergence of simple life cannot be excluded for other planets and moons in our solar system. However, this could not be proved so far.
The search for traces of fossil or recent life in the solar system will help to determine the factor \(f_l\) more precisely in the future. Currently, many authors assume that simple life could evolve as soon as it is physically and chemically possible.
$$\Large f_l \approx 1$$
If simple life emerged after 300 million years, why did the evolution to intelligent life take more than 4.5 billion years? Life remained unicellular for the first 2.5 billion years. If you could visit the Earth of that time, you would find a planet not unlike Mars, but with oceans, but Mars had those too in its early days. White clouds would pass through a shimmering orange, oxygen-poor atmosphere. There were few visible traces of life. One was stromatolites, limestone formations whose slimy surface was one of the few visible signs of microbial life.
2 billion years ago, the first cyanobacteria had begun to produce oxygen. Then, in Earth-historical dimensions only a little later, something happened that was to fundamentally change the further development of life. Somewhere, perhaps at the edge of a geothermal spring or in a tidal pool at the seashore, several bacteria entered into a symbiosis and formed a new cell type. More importantly, this new cell type was able to procreate and thus survived!
The transition from prokaryotes to eukaryotes was complete. In the cells, mitochondria now supplied the cell with energy, the nucleus contained the genetic material, and a cell membrane protected the new cell. A new type of unicellular life had emerged. Larger cells that could work more effectively through division of labor. On a geological scale, shortly thereafter, the first multicellular organisms emerged. A step in evolution that prokaryotes never succeeded in taking, but which has been independently demonstrated more than 25 times in eukaryotes.
From multicellular organisms vertebrates and plants should develop later, but the invasion of the land would have to wait for another billion years. Today one calls this time the "Cambrian explosion". In a short time span of 5 to 10 million years the precursors of all species known today developed. Every plant, every insect, every vertebrate that we know today or of which fossils have ever been found only came into being in the last 500 million years.
For the factor \(f_i\) this could mean that the formation of intelligent life takes a very long time. This is not certain, and there may well be good reasons why it has taken a long time on Earth. However, statistical studies suggest that it is more likely that \(f_i\) is significantly less than 1 [3].
$$\Large f_i << 1$$
A civilization will inevitably send signals into space at certain points in its technological development. This could be a conscious decision, but it is considerably more likely that they are technology signatures, such as radio waves, which are emitted only as a side effect.
The analog signals from Earth's first radio transmissions have now penetrated more than 100 light-years into space. However, since their transmission strength decreases with the square of the distance, they are likely to be very difficult to receive, if at all. In todays terrestrial radio transmissions, only a small amount of energy is radiated into space, because anything else would be a waste of energy, since all the receivers are located on Earth.
The time of strong radio emissions on earth is coming to an end. The frequencies are getting larger and larger in order to transmit data faster, the transmission power is getting smaller because the reception technology has developed further. Television towers are increasingly being replaced by geostationary satellites, which use much less transmitting power. If they still transmit, it is often with directional radio. Radio and television transmissions are increasingly being delivered via the Internet's cable networks. When terrestrial broadcasting actually still occurs, it is in compressed digital format that is difficult to distinguish from noise.
But not all communication is terrestrial. Once a civilization begins to explore its planetary system, it will need to communicate with its own spacecraft. For this type of communication, large radio antennas will be built to deliver their energy in a specific direction, and if the signals are delivered in pulse form, much higher transmission strengths can be achieved. The range of the signals is much greater, but they are sent only in the direction of the spacecraft and therefore can be received only on planetary systems behind it.
A direct message to extraterrestrial civilizations has been sent from Earth into space only once so far. In 1974, the Arecibo radio telescope sent the so-called "Arecibo message" in the direction of the globular cluster M13, 25000 light years away. It was more a symbolic act than a real communication attempt, because the whole transmission lasted only 3 minutes! [6]
The energy expenditure to actively send signals into space is enormous, the benefit questionable. In which frequency band and how should be sent? With radio waves or rather lasers? Furthermore, there could be good reasons why civilizations, despite existing capability, do not want to communicate or even actively obscure the technology signatures of their planet.
Any estimation of \(f_c\) remains pure speculation. However, it is plausible to assume that due to involuntary radio emissions alone, the probability is significant and greater than 0. However, the comparatively short range severely limits the receivability of the signals. The transmission and reception technology has made enormous progress in the last 50 years. By interconnecting several radio telescopes, it is now possible to create virtual antennas on a scale that seemed unthinkable a few years ago. In 2012, for the SETI project, several radio telescopes distributed around the globe were connected for the first time by means of Very Long Baseline Interferometry in order to examine the star Gliese 581, 20 light-years away, for radio emissions. Nothing was found. [8] Detection of faint signals from several light-years away is now, if not already within the realm of the technically possible, at least within the realm of the technically conceivable.
But radio emissions are not the only detectable technology signature. It is conceivable that future generations of infrared telescopes could directly detect the heat signature of cities on exoplanets. Better optical telescopes could detect space debris in orbit around a planet, or large-scale technological structures "parked" at Lagrangian points or in geostationary orbit around a planet. An optimistic upper limit for \(f_c\) is therefore assumed to be 0.6.
Perhaps it is more difficult than expected to detect the technology signatures of other civilizations. Most communication on Earth today is encrypted, compressed, and digital. To outsiders, it might sound like noise. In the original formulation of the Drake equation \(f_c\) was estimated to be 0.1 to 0.2. Therefore, 0.1 shall serve as a lower limit here.
$$\Large 0.1 < f_c < 0.6$$
Although actively transmitting civilizations are unlikely, it is reasonable to assume that a technological civilization in its immediate vicinity should be detectable only as a result of its involuntarily emitted signals. The total period of detectability depends on many factors. However, the lifetime of the technologically active civilization plays a crucial role.
How long does a technological civilization exist? The lifetime could be limited by natural disasters on a planetary scale. Asteroid impacts, neighboring supernovae, gamma-ray bursts, or increased stellar activity have the potential to adversely affect a civilization or all life on a planet. Technological civilizations may be able to protect themselves to some extent from such events, but Earth history shows that mass extinctions are not uncommon.
A study of 60 past civilizations on Earth concluded that the average lifespan of such a civilization is 420 years. [7] But how meaningful are such numbers? The decline of one civilization on Earth has usually been synonymous with the rise of another. Populations and technologies were often assimilated.
Should we not rather speak of "human civilization" in relation to the Earth? On our planet there have been traces of different cultures for at least 5000 years and a line of development that can be drawn from the cultures of early times to the present day. One sees them in different language families, in cultural and scientific traditions. Why do we calculate today worldwide with Arabic numerals and not with Roman numerals? Why are Latin letters even on Chinese car license plates? The cultures on earth do not exist in isolation, they build on each other. The "world culture" of today is a mixture of the accumulated traditions and experiences of different civilizations over a period of more than 5000 years.
Of these 5000 years, however, only the last 250 years since the beginning of the Industrial Revolution can be considered technological. During this time, mankind has managed to cause lasting damage to the vital ozone layer and to influence the climate several times. For example, severe air pollution in the second half of the 20th century led to a decrease in the amount of solar radiation reaching the Earth's surface. An effect called "Global Dimming" was so alarming to some researchers in the 1970s that they feared a man-made ice age. This effect masked the rise of greenhouse gases in the atmosphere and now that the atmosphere is cleaner in many places, we are seeing signs of global warming. Humanity has arrived in the Anthropocene, a (so far only proposed) geologic age in which humans have become the most important influence on the planet's ecosystem.
Perhaps the age of mankind as a technological civilization should only be given as 75 years. This is the period since the detonation of the first atomic bomb. A weapon with which it was possible for the first time to destroy the complete biosphere of the planet. Currently, 9 nations have an estimated total of 14000 nuclear warheads. More than enough to set back life on the planet by several 100 million years.
The optimistic assumption is that a civilization that has reached a certain level of technology can survive for a very long time. Mankind has proven that it can influence the earth's climate in one direction or another. A civilization could use this knowledge to specifically influence the climate by means of geoengineering. Be it to protect itself from the consequences of a self-made global warming or to neutralize the consequences of a changed energy output of its home star.
Even the use of nuclear weapons does not necessarily have to destroy a planetary civilization completely. On Earth, for example, the population is essentially concentrated in the northern hemisphere. Hydrogen bombs cause comparatively little radioactive fallout and the transfer of radioactive material from the northern to the southern hemisphere is rather small due to largely separated wind systems. Thus, only part of a planetary civilization could be affected by a "global" thermonuclear war.
An extensive destruction of the infrastructure of the northern hemisphere of the earth would not automatically mean that people in Africa, South America or Australia would not know how semiconductors or transformers work. Most production facilities would be destroyed, but the basic knowledge would remain. One would be able to produce computer technology on the level of the 80's also in Africa comparatively fast.
The optimistic estimate for the life span of a civilization is therefore "very long". It is conceivable that a technologically far developed civilization exists several thousand or even million years. If it is not able to leave its planetary system, the limited life span of its central star will finally put an end to it. If, however, it succeeds in leaving its own star, there would be practically no limits to its survival.
$$\Large L >> 1000$$
On Earth mankind could probably survive for another 500 to 600 million years. After that, Earth will be a hot, uninhabitable planet without water. At that time, Mars could offer the more life-friendly alternative for a high-tech civilization, because the sun's habitable zone will shift outward as solar activity increases.
There is evidence that Mars has not completely lost its oceans, but that they are merely frozen. If the habitable zone were to be extended to the orbit of Mars, they could thaw again and provide liquid surface water for at least several million years and create a denser atmosphere.
It is conceivable that characteristics necessary to make a species the dominant species of a planet are detrimental to its long-term survival. The pessimistic assumption is therefore that highly developed civilizations tend to self-destruct. If not by wars, then by destruction of their own ecosystem. Such a civilization endangers itself by discovering nuclear fission and nuclear fusion, is not able to control the negative consequences of its own technological development, and is on the verge of self-destruction in case of internal conflicts.
$$\Large L < 1000$$
There is little point in asking for the result of the Drake equation at this time. The result can be close to zero or show hundreds of millions of detectable civilizations, depending on the choice of parameters. But nobody comes to this website to read that. So let's calculate two example scenarios, one optimistic and one pessimistic.
In the optimistic scenario, we assume that 3 stars are formed per year, providing potentially life-friendly conditions. The emergence of intelligent life is virtually inevitable and half of all civilizations emit detectable signals. The civilizations have an average lifetime of one million years.
\begin{equation} \Large \nonumber N = 3.0 \cdot 1 \cdot 0.4 \cdot 1\cdot 0.5 \cdot 1000000 = 600000 \end{equation}In this scenario, there are currently about 600000 technologically contactable civilizations within the Milky Way.
In the pessimistic scenario, we assume that only 1.5 stars are formed per year that provide life-friendly conditions. The emergence of intelligent life takes a long time and happens only in one out of a hundred cases. Only 10% of technological civilizations emit detectable signals and the average lifetime of a civilization is only 1000 years.
\begin{equation} \Large \nonumber N = 1.5 \cdot 1 \cdot 0.4 \cdot 0.01 \cdot 0.1 \cdot 1000 = 0.6 \end{equation}According to this scenario, not even a single communicating technological civilization would exist within the Milky Way. We know that there is at least one, but statistically the result only means that intelligent life is extremely rare and in another, comparable galaxy no single civilization would exist.
This result does not exclude the possibility that there are thousands or even millions of other planets in the Milky Way where life exists, as long as it remains below the threshold for detectable technological civilization. Earth did not take this threshold until 100 years ago, when radio technology began to take hold. Taking a pessimistic view of the detectability of radio signals, we may still be below it. In such a scenario the only ever detectable communication attempt from Earth could have been the 3 minutes of the Arecibo signal.